Major changes in the secondary, tertiary, and quaternary structures without cleavage of backbone peptide bonds are regarded as “denaturation”. Subtle changes in structure, which do not drastically alter the molecular architecture of the protein, are usually regarded as “conformational adaptability”. Breakdown of peptide bonds of protein into peptides or amino acid is “digestion”
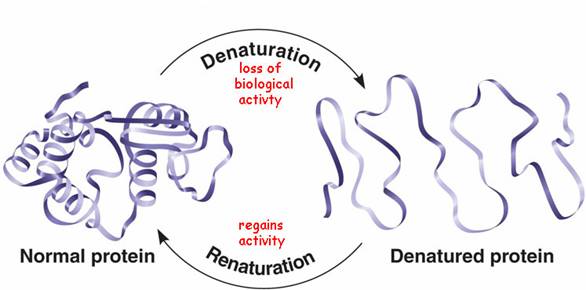
The structure of a protein depends on various attractive and repulsive interactions resulting from various intra-molecular forces as well as interaction of various protein groups with surrounding solvent water. The native state (of a single protein molecule) is thermodynamically the most stable with lowest feasible free energy at physiological conditions. Any change in its environment, such as pH, ionic strength, temperature, solvent composition, etc., will force the molecule to assume a new equilibrium structure.
Understanding protein structure
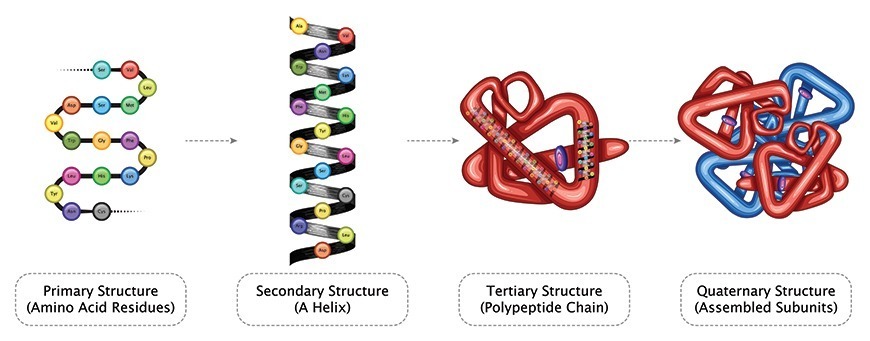
How do we measure protein denaturation
Denaturation involves transformation of a well-defined, folded structure of a protein, formed under physiological conditions, to an unfolded state under non-physiological conditions. As we know, we cannot measure structure directly i.e. direct measurement of the fractions of native and denatured protein in a solution is not possible. But, conformational changes in proteins invariably affect several of its chemical and physical properties, such as ultraviolet (UV) absorbance, fluorescence, viscosity, sedimentation coefficient, optical rotation, circular dichroism, reactivity of sulfhydryl groups, and enzyme activity. Thus, protein denaturation can be studied by monitoring changes in these physical and chemical properties.
Heat as denaturant
Heat is the most commonly used agent in food processing and preservation. When a protein solution is gradually heated above a critical temperature, it undergoes a sharp transition from the native state to the denatured state. The temperature at the transition midpoint, where t he concentration ratio of native and denatured states is 1,is known either as the melting temperature Tm, or the denaturation temperature Td.
The mechanism of temperature-induced denaturation is highly complex and involves primarily destabilization of the major non-covalent interactions. Hydrogen bonding, electrostatic, and van der Waals interactions are exothermic (enthalpy driven) in nature. Therefore, they are destabilized at high temperatures and stabilized at low temperatures. However, since peptide hydrogen bonds in proteins are mostly buried in the interior, they remain stable over a wide range of temperature. On the other hand, hydrophobic interactions are endothermic (entropy driven). They are stabilized at high temperatures and destabilized at low temperatures. Therefore, as the temperature is increased, the changes in the stabilities of these two groups of noncovalent interactions oppose each other. However, the stability of hydrophobic interactions cannot increase infinitely with increasing temperature, because above a certain temperature, gradual breakdown of water structure will eventually destabilize hydrophobic interactions as well. The strength of hydrophobic interactions reaches a maximum at about 60–70°C.
Another major force that affects conformational stability of proteins is the conformational entropy, –T DSConf, of the polypeptide chain. As temperature is increased, the increase in thermal kinetic energy of the polypeptide chain greatly facilitates unfolding of the polypeptide chain.
Protein denaturation and amino acid composition
Protein denaturation by heat also depends on the amino acid composition of proteins. Proteins that contain a greater proportion of hydrophobic amino acid residues, especially Val, Ile, Leu, and Phe, tend to be more stable than the more hydrophilic proteins. Proteins of thermophilic organisms usually contain large amounts of hydrophobic amino acid residues. It is also said that other factors, such as disulfide bonds and the presence of salt bridges buried in hydrophobic clefts, may also contribute to thermostability.
Protein denaturation and water content
Water greatly facilitates thermal denaturation of proteins [37,95]. Dry protein powders are extremely stable to thermal denaturation. The value of Td decreases rapidly as the water content is increased from 0 to 0.35 g water/g protein. The effect of hydration on thermostability is fundamentally related to protein dynamics. In the dry state, proteins have a static structure, that is, the mobility of polypeptide segments is restricted. As the water content is increased, hydration and partial penetration of water into surface cavities cause swelling of the protein. The swelling of the protein increases chain mobility and flexibility, and the protein molecule assumes a more dynamic molten structure. When heated, this dynamic flexible structure provides greater access of water to salt bridges and peptide hydrogen bonds than is possible in the dry state, resulting in a lower Td.
Cold induced denaturation
It is said that the lower the temperature, the greater will be the stability of a protein. There are exceptions to this rule. Those proteins in which polar interactions dominate over nonpolar interactions are more stable at or below refrigeration temperatures than they are at higher temperatures. On the other hand, proteins that are primarily stabilized by hydrophobic interactions are more stable at about ambient temperature than they are at refrigeration temperature.
- The stability of lysozyme increases with lowering of temperature, whereas those of myoglobin and a mutant phage T4 lysozyme show maximum stability at about 30°C and 12.5°C, respectively. Below and above these temperatures, myoglobin and T4 lysozyme are less stable. When stored below 0°C, these two proteins undergo cold-induced denaturation.
- When skim milk is stored at 4°C, b-casein dissociates from casein micelles, and this alters the physicochemical and rennetting properties of the micelles.
Protein denaturation is reversible.
When the denaturant is removed from the protein solution (or the sample is cooled), most monomeric proteins (in the absence of aggregation) refold to their native conformation under appropriate solution conditions, such as pH, ionic strength, redox potential, and protein concentration.
- Glycinin, one of the storage proteins of soybean, aggregates and precipitates when stored at 2°C, then becomes soluble when returned to ambient temperature.
- Several oligomeric enzymes, such as lactate dehydrogenase and glyceraldehyde phosphate dehydrogenase, lose most of their enzyme activity when stored at 4°C, and this has been attributed to dissociation of the subunits. However, when warmed to and held at ambient temperature for a few hours, they reassociate and completely regain their activity
- Thermal denaturation of monomeric globular proteins is mostly reversible. For example, when many monomeric enzymes are heated above their denaturation temperatures, or even briefly held at 100°C, and then are immediately cooled to room temperature, they fully regain their activities. However, thermal denaturation can become irreversible when the protein is heated at 90–100°C for a prolonged period even at neutral pH. This irreversibility occurs because of several chemical changes in the protein, such as deamidation of Asn residues, cleavage of peptide bonds at Asp residues, destruction of Cys and cystine residues, and aggregation.
Suggested lecture on Protein denaturation
Reference : Food Chemistry l1996 l 3rd Ed l By O R Fennema l Marcel Dekker
Simplified and accurate